Accuracy and safety of deep brain stimulation assisted by a neurosurgical robot attachable to the operating table
Article information
Abstract
Objective
Neurosurgical robots have recently been introduced to assist in guiding trajectories to achieve accurate targeting. This study investigated the accuracy of robot-assisted deep brain stimulation (DBS) compared with conventional DBS using the Leksell arc system.
Methods
Between October 2020 and September 2021, 29 patients underwent DBS using the Leksell arc system, while from October 2021 to August 2022, 20 patients underwent DBS using a neurosurgical robot system (KYMERO; Koh Young Technology). Radial errors, total operative time (surgery time, preparation time), and clinical outcomes were compared between both groups through a retrospective analysis.
Results
Radial errors were significantly smaller in robot-assisted DBS (1.63±0.39 mm vs. 1.01±0.39 mm, p<0.01). Furthermore, the error of the second implantation was smaller than in the Leksell DBS group (0.216 vs. 0.005 mm, p<0.001). The total operative time of DBS with Leksell frame was 3.47 hours (range, 2.38–5.85 hours) and the surgery time was 2.21 hours (range, 1.08–4.67 hours), while the total operative time in the robot group was 3.92 hours (range, 2.75–5.85 hours) and the surgical time was 2.60 hours (range, 1.02–4.67 hours) (p=0.055, p=0.258, respectively). The clinical outcome did not show a significant difference according to the surgical method.
Conclusion
DBS with a robot-assisted system enables the accurate and consistent insertion of DBS electrodes with a lower error rate than the conventional method.
INTRODUCTION
The use of robots in neurosurgery is more restricted compared to other surgeries within the abdominal or thoracic cavity, due to the requirement for precise control of instruments in a limited space. The first reported use of robots in neurosurgical procedures was the PUMA 560 robotic system, which was introduced in 1985, to perform biopsy [1] which guided the trajectory of the needle without errors from the hand tremors of the surgeon. Since then, many types of robots have been developed, especially for stereotactic procedures that require highly precise targeting with minimal error. More recently, the use of robots has increased, and many products are commercially available [2].
Deep brain stimulation (DBS) is a widely accepted procedure for controlling movement disorders, Parkinson’s disease, dystonia, and essential tremor. Other targets for the treatment of intractable epilepsy, cluster headaches, and many other disorders related to diverse neural circuits are under investigation. DBS requires high-precision targeting for implanting an electrode into the sweet spot inside the targeted nuclei by only few millimeters.
We recently adopted the KYMERO robot-assisted stereotactic surgical system (Koh Young Technology) which can be attached to the operating table. We report our experiences with robot-assisted DBS and compare the accuracy of DBS electrode placement using conventional Leksell frame-based and robot-assisted surgeries.
MATERIALS AND METHODS
Forty-nine patients who underwent DBS at a single center by a single neurosurgeon between October 2020 and August 2022 were retrospectively reviewed. From October 2020 to September 2021, a total of 29 patients underwent DBS using the Leksell arc system. After the introduction of the KYMERO in our center in July 2021, from October 2021 to August 2022, a total of 20 patients underwent robotic surgery.
Data on basic demographics, targeted nucleus, diagnosis of DBS implant, implantation method using Leksell stereotactic arc or robotic system, total operative time (TOT) for patient anesthesia, and surgery time (ST) were collected from electronic medical records. The basic demographics of the patients in each surgical groups are shown in Table 1.
Ethical statements
This retrospective study was approved by institutional review board of the Samsung Medical Center (File no. : 2022-11-059-002). Informed consent was taken from every patients in this study.
Surgical procedure
Classic Leksell arc method
The Leksell frame was fixed on the patient’s head under local anesthesia. In early surgeries, we then took a 1.5-T magnetic resonance imaging (MRI) with markers attached to the frame. However, in most cases in this study, 3-T MRI was performed previously, and we performed intraoperative computed tomography (CT) with marker attached to the frame and then fused it in a stereotactic surgical targeting system such as Stealthstation (Medtronic). In all cases, we implanted the electrode on the left side first, followed by the right side. We placed a bilateral C-shaped incision, and burr hole was drilled using a 14 mm perforator drill. After setting x, y, and z from the Leksell system, the dura was incised and the sulcus and cortical vessels were avoided. Depending on the target, microelectrode recording is generally taken in a single trajectory and checked using a C-arm fluoroscope to confirm that the tip is located at the right target. A permanent electrode was then inserted, and macrostimulation was tested in awake surgery cases. The same procedures were performed on the other side when implanted bilaterally, and crosshair markers were used to check whether both electrodes were implanted symmetrically without significant errors.
The surgical method with a robotic system
The basic surgical tactics did not differ from the classic method. The O-frame, which is compatible with the KYMERO system, was fixed on the patient’s head in the operating room. Subsequently, an intraoperative CT scan was performed and fused with a preoperative MRI. After planning the trajectory and targeting the implant electrodes, the robot was attached to the operation table and entry point was marked using a laser guide. Unlike other stereotactic robots used to guide the trajectory, KYMERO can modify the plan by changing the entry or target point during the surgery. The entry was carefully modified to avoid obstacles from the skull edge and cortical vessels from the originally planned trajectory. After confirming the final trajectory, macrostimulation were tested for awake surgery cases and routine DBS procedures were performed. KYMERO provided a crosshair system, such as the Leksell arc system to check symmetricity; thus, confirmation was performed with the C-arm after implantation. Surgical flow of KYMERO robotic stereotactic surgery is described in Fig. 1.

(A) An O-frame is fixated to the patient’s head and intraoperative computed tomography is taken. (B) The stereotactic images are fused with preoperative magnetic resonance imaging, and planning is done with planning software. (C) The KYMERO robot device is attached to the operating table. (D) The entry and trajectory are marked with a laser guide as set from the planning software. (E) After incision, the entry can be easily modified without modifying the entire plan. (F) Similar to the crosshairs in the Leksell arc system, KYMERO can confirm that the implant is well placed at the planned target with the KYMERO crosshair tool.
We usually implanted electrodes from Medtronic, 3389 or Boston scientific, DB-2202-45 for subthalamic nucleus (STN) DBS. We also used Medtronic, 3387 or St. Jude 0.5 mm for other cases, such as globus pallidus interna or ventralis intermedius nucleus of the thalamus (VIM) DBS.
After electrode implantation using both methods, mobile CT was performed to confirm that there were no immediate complications or misplacement, and an implantable pulse generator was inserted immediately electrode implantation under general anesthesia.
Measuring errors of the target
After implantation of the electrodes, the skin was temporarily closed, and the patient underwent intra-operative CT to check for any immediate complications and confirm electrode implantation at the intended target. Then, the postoperative CT images were fused with the planned target, and error between the planned target and final implantation was compared. Both the depth and radial errors of the implanted electrodes were measured. The depth error could be neglected. We generally put slightly deeper than the targeted point because we can have a longer range of stimulation, and when using the segmented electrode, locating the second of the third electrode from the distal end of the target is better to stimulate with directional modification. The main error that we measured was the radial error, which was calculated as the width between the intended target and final target point perpendicular to the intended trajectory, which was presented by Zrinzo [3]. We measured the distance between the intended target and center of the implanted electrode at the plane perpendicular to the trajectory of the implanted electrode (Fig. 2).

Schematic illustration of measuring radial errors (REs). (A) The black dot represents the intended target. RE is measured at the perpendicular plane, as the length between the intended target and the center of the electrode. (B) After implantation of the electrodes, intraoperative computed tomography is performed, and the image is fused with the planned image. The original trajectory and target are indicated by blue and red lines, respectively. (C) In the plane perpendicular to the electrode, the distance between the center of the electrode (black arrow) and intended target (white arrow) is measured. L.STN: left subthalamic nucleus.
Length of surgery and the learning curves
The TOT was calculated as the time between initiation of anesthesia, general anesthesia, or sedation with propofol during awake surgery, and the time of termination of anesthesia. The ST was calculated by making an incision until the wound was closed. The TOT and ST were calculated by investigating the time required for the insertion of intracranial electrodes and excluded the time for the insertion and connection of the implantable pulse generator. The time between TOT and ST was considered the preparation time (PT). We compared the TOT, ST, and PT of both surgical methods.
The analysis of surgical time excluded three patients from the Leksell group and four patients from the robot group who underwent unilateral electrode implantation. To determine the learning curves for handling the robotic system, the TOT and ST in the early and late halves of the robotic system were compared.
Clinical outcome
Clinical outcomes were measured differently for each diagnosis. Movement Disorder Society-Unified Parkinson Disease Rating Scale (UPDRS) part III was used for patients with idiopathic Parkinson’s disease (IPD), and the preoperative UPDRS score was measured in the medication-off state. After DBS implantation, DBS was turned on after a few months, owing to the lesion effect. The UPDRS III score was measured when DBS was turned on with the modification of medication. The best score measured during follow-up was counted. The Burke-Fahn-Marsden Dystonia Rating Scale (BFMDRS) was used for patients with dystonia and the Clinical Rating Scale for Tremor was used for patients with essential tremors. In other entities, the scale for clinical outcomes was followed. Pre- and postoperative scales were assessed and compared. The subjective outcomes were also assessed.
RESULTS
Radial error measurement
In the Leksell group, the mean radial error per lead was 1.63±0.39 mm (left side 1.54 mm and right side 1.72 mm) compared to 1.01±0.39 mm (left side 1.01 mm and right side 1.02 mm) in robot group. The radial error measured on each side was significantly smaller in the robot-assisted group (left, p=0.01; right, p<0.01) (Fig. 3).

Radial errors measured for left- and right-side implants using different surgical methods. On the left side, the Leksell group shows a 1.54 mm error, while the robot group shows 1.01 mm. Right-side implantation shows a 1.72 mm radial error in the Leksell group compared to the 1.02 mm error in the robot group. On each side, the difference is statistically significant (box=mean value±standard deviation, bold line=median value, vertical line=maximum and minimum values).
We also compared the differences between the first and second electrode implantation. In the Leksell group, the error in the second implantation was 0.216 mm larger than in the primary implantation. However, in the robot group, the second implant showed a 0.005 mm less error than the primary implantation.
When we stratified radial error by anesthesia selection, awake surgery might have more errors than asleep surgery because the brain might shift more during patient manipulation. However, there were no differences between asleep and awake DBS (1.26 mm vs. 1.48 mm, p=0.251). Moreover, the results were similar in each surgical method; the Leksell group had 1.56 and 1.69 mm errors (p=0.633) and the robot group had 0.92 and 1.10 mm errors (p=0.179) when asleep and awake, respectively.
Operation time
Mean TOT and ST was 3.47 hours (range, 2.38–5.85 hours) and 2.21 hours (range, 1.08–4.67 hours) in Leksell group compared to 3.92 hours (range, 2.75–5.85 hours) and 2.60 hours (range, 1.02–4.67 hours) for the robot group (p=0.055 and 0.258, respectively). Although surgery using a robot took more time for preparation of the robot, there were no significant differences in TOT, ST, and PT using the surgical method. In the early periods using robots, handling the robot, and planning using their software was not familiar, and thus took time to get used to it. In the early half of the cases, the TOT and ST took 4.20 and 2.46 hours, respectively; thus, the PT was 1.74 hours. In the last half of the cases, TOT decreased to 3.64 hours and ST was 2.74 hours, and PT decreased to 0.90 hours, which was significantly decreased in the early half of the cases (p<0.001). The time required for robot preparation and surgery was shortened by experiencing more cases with the robot (Fig. 4, 5).

Comparison of the surgery time and preparation time in Leksell-based surgery and robot-assisted surgery. The was no significant time difference between two groups (p=0.06). However, comparing the early half and later half of cases with the robot, the time for preparation was significantly reduced (p<0.001).
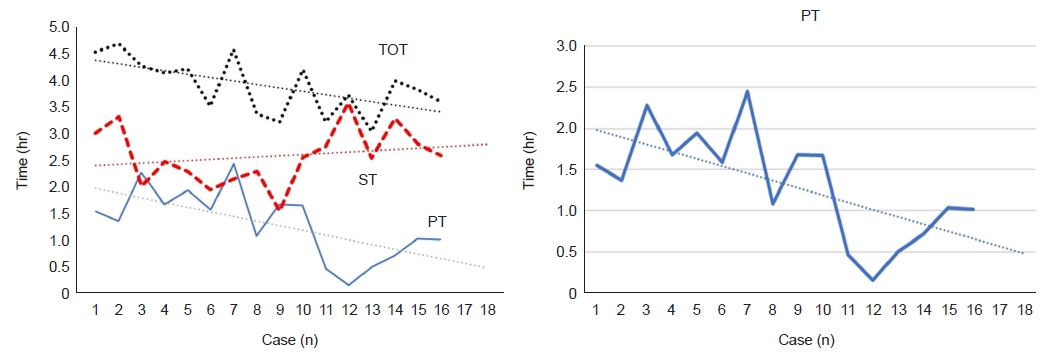
Preparation time (PT), total operative time (TOT), and surgery time (ST) were not significantly different between the Leksell-based surgery and robot-assisted surgery groups. However, comparing the early and late experiences with the robot, TOT and PT decreased as experience accumulated. PT showed a decrease from 1.74 to 0.90 hours in the early and late halves of the robot-assisted cases (n=16).
ST showed an increase in the latter half compared to the early half; however, the difference was not statistically significant (p=0.42).
We stratified operative time by anesthesia selection and made a comparison between the robot and the Leksell group. Mean TOT and ST was 4.01 and 2.58 hours in the Robot group while surgery was performed under awake anesthesia compared to 3.32 and 2.12 hours in the Leksell group performed under awake anesthesia (p=0.014 and 0.08, respectively). Mean TOT and ST was 3.77 and 2.55 hours in the Robot group while surgery was performed under general anesthesia compared to 3.50 and 2.27 hours in the Leksell group performed under general anesthesia (p=0.71 and 0.42, respectively). Only TOT showed significantly shorter in Leksell group when performed under awake surgery.
Clinical outcome
The postoperative mean follow-up period was 4.1±3.0 months (range, 1–9 months) for Leksell group and 1.8±2.6 months (range, 1–11 months) for robot group. In patients with IPD, the UPDRS III score measured in the Leksell group at the preoperative off state was 55.33±13.99 and improved to 16.40±9.85 after surgery with or without medication. In the robot group, the preoperative UPDRS score was 49.91±15.88 and improved to 11.55±6.85. The mean improvement in the UPDRS score and ratio of improvement was 38.93, 38.36 (p=0.93) and 71.17% and 73.37% (p=0.77) in the Leksell and robot groups, respectively. There was no statistical difference in clinical outcomes using the classic Leksell arc or KYMERO robotic system.
Except for DBS implanted for patients with IPD, the number of cases was too small for statistical analysis. The mean BFMDRS score before DBS was 53.75 and improved to 34.00 in four patients with dystonia. There were six essential tremor patients, three implanted at the bilateral VIM, two at the unilateral VIM, and one at the left posterior subthalamic area (PSA). Four patients in the Leksell group improved their tremor scale from 42.59 to 25.00, while only one patient in the robot group showed improvement from 66 to 40. However, one patient implanted with PSA who had the previous failure of VIM DBS had less effect on dysphonia complications and had no improvement after repositioning of the electrode.
Six DBS implants were implanted in patients with epilepsy, five at the bilateral anterior thalamic nucleus, and one at the bilateral centromedian thalamic nucleus. Five patients implanted and electrode with a Leksell system, and one with a robot. The median frequency of seizures was reduced by 80% from that before DBS in four patients. One patient had reduced severity, however, no reduction in frequency. In one patient where an electrode was implanted using a robot, a radial error of 1 mm was observed; however, he did not show efficacy till 6 months after follow-up.
One patient with intractable cluster headache who had a previous DBS implant in the left ventral tegmental area (VTA) was again implanted at the contralateral side of the VTA, and the numeric pain score reduced from 9 to 6.
Postoperative complications
In all 49 patients DBS was successfully performed (91 leads). Serious postoperative complications such as intracranial bleeding were not observed.
DISCUSSION
After selecting the right candidates for DBS and target for stimulation, the matter of concern for the surgeon is to place the electrode at the intended target as accurately as possible [4]. There are many factors influencing the misplacement of the electrode, which account for 46% of treatment failures that require revision surgery [5-7]. The limit of errors to achieve clinical benefit in STN DBS was considered to be less than 3 mm of the intended target [8,9]. However, in general, the accepted accuracy is less than 2 mm in recent studies, including meta-analyses of radial errors and clinical outcomes [10,11]. Furthermore, the recent use of directional electrodes might modify the stimulated area more precisely, thus compensating for implant errors. In this study, regardless of different targets for diverse indications, mean radial errors were less than 2 mm in both the robot and Leksell groups, and there was no significant difference in clinical outcomes between the two groups. Although there are few clinical differences, the goal for electrode implantation should be the accurate placement of the electrode at the intended point with minimum error. With the advancement of imaging techniques, 7-T MRI, diffusion tractography, and functional imaging, we can seek a new target to be stimulated, which is yet to be unveiled. Thus, the consistent accuracy of implants is always a matter for stereotactic surgery.
In recent reports of errors achieved with other robot-assisted DBS, mean radial errors ranged from 0.6 to 1.7 mm and most studies comparing errors with the classic method showed better non-inferior outcomes in robot groups; the errors from other robot-based DBS surgeries are shown in Table 2 [12-25].
Fenoy and Conner [13] reported there were larger errors in robot-assisted DBS compared to the classic frame-based group, 0.98 and 0.74 mm for robot-assisted and Leksell groups, respectively; however, the error they reported was much smaller than that reported in previous studies. The placement of the second electrode may have a larger error than that of the primary implant. Bunyaratavej et al. [26] reported that the error was significantly larger for the second implant, usually in the antero-posterior direction. Liang et al. [19] also reported error of the second implant (right side) was much larger than that of the first implant (left side) when using frameless robot system. The error might be a consequence of brain shift that results from cerebrospinal fluid (CSF) loss and pneumocephalus that occurs after surgery, especially after more manipulation of patients with macrostimulations [27,28]. We evaluated the error between the planned target and implanted electrode tip by co-registering the preoperative planning images with immediate postoperative CT images. The immediate postoperative CT images reflect both the targeting error and brain shift. Precisely calculate the degree of contribution of CSF loss and pneumocephalus to the brain shift is difficult and this is a limitation of our study.
We used a robot attached to the operation table, and the head was fixed to the frame. This robot system minimizes the error derived from the angular movement of the robot arm. Also, this robot system enables flexible modification and automatic calculation of the trajectory. Otherwise, conventional Leksell frame method requires manual setting of x, y, and z coordinates. The difference in this process may reduce the potential for human error to submillimeter levels in robot-assisted surgery system, and we achieved significantly lower error compared to the classical Leksell frame method.
In recent years, with advances in imaging techniques, more accurate planning has become possible; thus, asleep DBS has been widely performed. Asleep DBS has several advantages for both patients and surgeons. One of disadvantage of asleep DBS is surgeon cannot convince implant to be placed at right “functional target.” Thus, many tracts of electrophysiologic mapping were performed to ensure that the target was truly intended and to modify the trajectory to reduce the error. However, recording multiple tracts to confirm the target may be related to the increased error of the second implant [29] and increase the risk of hemorrhagic complications and longer operation time, which might increase the error from brain shift [30]. Hence, intraoperative imaging and improvement in stereotactic techniques may reduce the need for electrophysiological mapping [30]. We could achieve a submillimeter error from the robot-assisted surgery. Moreover, the error between the first and second implantations was significantly low. This consistent result may provide confidence to the surgeon and reduce the number of electrophysiological recordings during implantation surgery. Consequently, this might reduce the operation time and minimize the chance of an increase in the second implant error.
The early practice of new techniques is time-consuming. The robot gives more accurate targeting with consistent results than the manual target; however, more time is required in setting the robot and additional intraoperative images should be taken for the robot registration or confirming the implant placement at the right target [13,23]. Functional neurosurgeons who perform DBS are familiar with setting x, y, and z coordinates from their commonly used frame system, for example, Leksell G-frame and Cosman-Roberts-Wells frame; however, new frame or frameless robot systems are difficult to handle in early periods. We also took longer to prepare the robotic system, and the surgery took longer in the early periods. However, in cases held in the latter half, the time for robot preparation was significantly reduced and was not different from classic Leksell-based surgery. When comparing the operative time for each surgical method according to the anesthesia technique, the Leksell group demonstrated a shorter TOT than the robot group. The reason for this could be that the majority of awake surgeries were performed during the early half, a period which required more time for surgical preparation.
KYMERO presents a workflow highly similar to that of the conventional frame-based surgery, which is a bedside attached device that is more familiar with the surgeon’s position during surgery. The most distinguishing factor from other stereotactic robots is the cross-hair system, which can easily confirm the symmetricity without intraoperative CT or MRI, as well as confirm the target point with the cross-hair as routinely held in the classic Leksell arc-based method. In other surgical robots, intraoperative imaging is mandatory to confirm whether the implant is right at the target. Taking an intraoperative CT scan and imaging fusion take more time than fluoroscopic imaging using a C-arm. The use of a cross-hair system to confirm the target may reduce ST, results in more consistent results, and minimize errors with robotic surgery.
Limitations
This is a retrospective, single-institution study without randomization. Therefore, the study design has inherent limitations. Our study is a preliminary report of the initial experience of robot-assisted DBS utilized KYMERO. Further studies with a larger number of patients and long-term outcomes would be required in future.
CONCLUSION
We achieved significantly less error from robot-assisted surgery, with highly consistent results between bilateral electrode implantations. We needed learning curves to use these new mechanics and unfamiliar procedures; however, PT reduced significantly after a few cases were experienced, and the time for surgery was not longer than that of previous methods. With this robotic system, we could achieve more accurate targeting and be more confident in sleep surgeries.
Notes
CONFLICTS OF INTEREST
No potential conflict of interest relevant to this article was reported.